A model for malaria treatment evaluation in the presence of multiple species
Abstract
Plasmodium falciparum and P. vivax are the two most common causes of malaria. While the majority of deaths and severe morbidity are due to P. falciparum, P. vivax poses a greater challenge to eliminating malaria outside of Africa due to its ability to form latent liver stage parasites (hypnozoites), which can cause relapsing episodes within an individual patient. In areas where P. falciparum and P. vivax are co-endemic, individuals can carry parasites of both species simultaneously. These mixed infections complicate dynamics in several ways: treatment of mixed infections will simultaneously affect both species, P. falciparum can mask the detection of P. vivax, and it has been hypothesised that clearing P. falciparum may trigger a relapse of dormant P. vivax. When mixed infections are treated for only blood-stage parasites, patients are at risk of relapse infections due to P. vivax hypnozoites.
We present a stochastic mathematical model that captures interactions between P. falciparum and P. vivax, and incorporates both standard schizonticidal treatment (which targets blood-stage parasites) and radical treatment (which additionally targets liver-stage parasites). We apply this model to assess the implications of mass drug administration (MDA), different treatment coverage of radical cure for mixed and P. vivax infections and a “unified radical cure” treatment strategy where P. falciparum, P. vivax and mixed infections all receive radical cure after screening glucose-6-phosphate dehydrogenase (G6PD) normal. We find that a unified radical cure strategy leads to a substantially lower incidence of malaria cases and deaths overall. MDA with schizonticidal treatment was found to decrease P. falciparumwith little effect on P. vivax. We perform a univariate sensitivity analysis to highlight important model parameters.
keywords:
Malaria , Unified treatment , Plasmodium falciparum , Plasmodium vivax , Stochastic modelling∗ Corresponding author: camelia.walker@unimelb.edu.au
- P.
- Plasmodium
- P. falciparum
- Plasmodium falciparum
- P. vivax
- Plasmodium vivax
- Po
- Plasmodium (P.) ovale
- Pm
- P. malariae
- Pk
- P. knowlesi
- ACT
- artemisinin-based combination therapies
- CQ
- chloroquine
- An.
- Anopheles
- CTMC
- continuous-time Markov chain
- FSAT
- focal screening and treatment
- G6PD
- glucose-6-phosphate dehydrogenase
- GMS
- Greater Mekong Subregion
- MDA
- mass drug administration
- ODE
- ordinary differential equation
- RDT
- rapid diagnostic test
1 Introduction
Almost half of the world’s population is at risk of malaria, with ongoing transmission reported in 85 countries [1]. In 2020 there were an estimated 241 million cases and 627,000 malaria deaths, with funding for control and elimination estimated at US$3.3 billion [1]. Over the last decade substantial gains have been made in reducing the burden of disease. In 2014 the leaders of 18 malaria endemic countries in the Asia Pacific committed to eliminating the disease in the region by 2030 [2]. In this region the two parasite species that cause the greatest burden of malaria are Plasmodium falciparum and P. vivax. Most research and intervention efforts have been focused on P. falciparum, the most pathogenic parasite species. However, outside of Africa P. vivax is becoming the predominant cause of malaria, and almost invariably co-exists with P. falciparum. While malaria control measures impact both species, these are often less effective against P. vivax primarily due to the parasite’s ability to form dormant liver parasites (hypnozoites) that can reactivate weeks to months after the initial infection, causing future infections (relapses). P. vivax also forms sexual stages early in infection and is able to transmit to the mosquito vector before the patient seeks treatment. Furthermore, P. vivax’s lower parasite density makes it more difficult to detect than P. falciparum.
Primaquine is the only widely-used drug available that kills hypnozoites. The combination of primaquine plus a schizonticidal drug, such as chloroquine (CQ) or artemisinin-based combination therapies (ACT), is known as radical cure. Primaquine can cause drug-induced haemolysis in individuals with glucose-6-phosphate dehydrogenase (G6PD) deficiency, an inherited enzymopathy present in up to 30% of malaria endemic populations. For this reason the WHO recommends screening for G6PD deficiency prior to administration of primaquine to reduce the risk of severe primaquine-induced haemolysis [3]. The effectiveness of primaquine is limited by the reluctance of healthcare providers to prescribe it, and patient adherence to complete a course of treatment [4]. New point-of-care tools for diagnosing G6PD deficiency have recently come onto the market but have yet to be introduced widely into clinical practice. The challenges in safely and consistently treating P. vivax with radical cure has resulted in its relative rise as a proportion of malaria cases [5, 6, 7]. In one modelling study over 80% of P. vivax cases in the Greater Mekong Subregion (GMS) was estimated to have arisen from relapses [8], highlighting the importance of radical cure to reduce the burden of disease [9].
Successful malaria elimination campaigns in co-endemic settings will require widespread use of safe and effective radical cure to patients presenting with P. vivax as well as the hidden reservoirs of infection. Failure to consider P. vivax malaria as a target for elimination may compromise P. falciparum elimination campaigns because communities that continue to experience cases of malaria, even if due to another type of parasite, may show a reduced willingness to participate in future interventions designed to prevent re-introduction of P. falciparum. In an effort to accelerate P. falciparum malaria elimination in the GMS, the use of mass drug administration (MDA) or mass screening and treatment is now being investigated [10]. These interventions do not include radical cure, but all stages (blood and liver) of all parasite species will need to be eradicated to eliminate malaria.
Cambodia aims to eliminate all species of malaria by 2025. In 2019, mixed infections of both P. falciparum and P. vivax accounted for 16.6% of malaria infections [11]. Mixed infections can change treatment outcomes in several ways: a mixed infection will be treated for both species simultaneously, P. falciparum malaria can mask a P. vivax malaria co-infection [12, 13] and an episode of P. falciparum malaria is associated with a greater risk of P. vivax infection in the subsequent weeks after treatment [14, 15, 16]. It has been hypothesized that the fever and haemolysis caused by acute P. falciparum malaria may trigger reactivation of P. vivax hypnozoites and subsequent relapse. Whereas current radical cure policy is reserved for patients presenting with P. vivax malaria, a unified treatment policy, in which patients presenting with either P. vivax or P. falciparum are prescribed radical cure, has potential to reduce recurrent episodes of malaria and target hidden reservoirs of infection [17].
While a range of mathematical models for malaria have been proposed, implemented, analysed and used to support policy decisions over the last 100 years—as reviewed recently [18, 19]—few models have included the parasite dynamics of both P. falciparum and P. vivax [20, 21, 22, 23]. To our knowledge, only one of these modelling investigations explored interactions between species [23]. Shretta et al. developed a deterministic metapopulation model of P. falciparum and P. vivax, and incorporated key interactions between P. falciparum and P. vivax, including “treatment entanglement” (any treatment affecting the other parasite species), “triggering” (P. vivax hypnozoite activation following an episode of P. falciparum), and “masking” (where non-P. falciparum rapid diagnostic test (RDT) results are either missed or falsely attributed to be P. falciparum). The remaining models [20, 21, 22] explicitly and/or effectively consider the dynamics of the two species to be completely independent.
We present the first stochastic agent-based model for the transmission of both P. falciparum and P. vivax, which addresses the dynamics of mixed infections, parasite interactions and antimalarial treatments. Our model considers humans as discrete agents which transition between compartments according to a continuous-time Markov chain (CTMC) model. The CTMC is coupled with a system of ordinary differential equations that govern the mosquito population, where the transmission rate both from mosquitoes-to-humans and humans-to-mosquitoes are held constant over small time-steps. The stochasticity of our model allows it to appropriately capture infectious disease dynamics at low incidence, as malaria approaches elimination. Our model has 6 compartments for P. falciparum and 7 for P. vivax, representing a model with lower complexity than the other multi-species model with interactions, which has 14 and 17 compartments, respectively [23]. The reduction in model complexity partially comes from removing age-stratification from the model. One of the main effects of age is in the acquisition of immunity to prevent developing clinical malaria, which we capture through lower probabilities of clinical malaria upon reinfection or relapse (i.e., in the following, the probability of clinical malaria upon reinfection or relapse is 0.5, compared to 0.95 for a naive P. falciparum infection). Even with the reduction in model complexity compared to [23], we note that our model requires many input parameters, many of which are poorly defined in the literature. Hence, we perform a univariate sensitivity analysis to understand the impact of changes to each parameter with respect to the model outputs, malaria cases and deaths.
As an example, we consider scenarios with Cambodia-like P. falciparum and P. vivax prevalence and parameters, since both P. falciparum and P. vivax are present and the Anopheles (An.) populations are able to transmit both. Our model is applied to assess the effect of blood-stage treatment, differing coverage of radical cure prescription, and a unified treatment policy in which radical cure is prescribed to patients presenting with P. vivax, P. falciparum and mixed infections. For each of these treatment scenarios, we also consider an MDA intervention, where a proportion of the population are prescribed blood-stage treatment, which allows for asymptomatic infections to be treated.
2 Methods
2.1 Transmission model
To capture the transmission dynamics of both P. falciparum and P. vivax, we use a stochastic agent-based approach for the human population coupled with a deterministic system of ODEs for the mosquito population. Each human agent has their status with respect to both P. falciparum and P. vivax tracked over time, which allows mixed infections to be captured. The agent-based model is implemented by holding rates constant over discrete time-steps for computational efficiency and for ease of coupling to the ODEs that govern the mosquito population.
Each individual’s state is bivariate to specify their state with respect to P. falciparum and to P. vivax. For each Plasmodium species, humans are regarded as being susceptible (), infectious with clinical symptoms (), infectious but asymptomatic (), recovered with no hypnozoites (), recovered with hypnozoites ( for latent: not applicable for P. falciparum), undergoing blood-stage treatment with no radical cure (), or undergoing treatment with radical cure (). Radical cure is defined as low-dose primaquine (3.5mg/kg total) administered over 14 days. A simplified schematic of the human transitions are depicted in Figure 1. Given the large number of connections between states required to describe the transmission and treatment dynamics, the model schematic uses a single line between connectors where multiple transitions apply and does not depict interactions between the species. The full list of possible transition rates and stoichiometries is provided in the Supplementary Table S1.
The dynamics of a human individual infected with type malaria are briefly described here for (P. falciparum) and (P. vivax). Individuals susceptible () to type malaria are infected at rate . Upon infection they develop clinical symptoms () with probability or are otherwise asymptomatic (). Individuals are symptomatic for a mean duration of , at which point they either become asymptomatic () or die without treatment with probability . The individuals with clinical symptoms may be treated at rate , where is the probability that they are able to access healthcare and is the rate at which medical attention is sought if it is readily available. Individuals with asymptomatic malaria will clear all blood-stage parasites at rate and, for P. vivax, will be left with hypnozoites with probability . When an individual with P. vivax seeks treatment they are prescribed radical cure () with probability , otherwise they receive blood-stage treatment ().
Any infectious individual may additionally be treated at rate via an intervention program (such as MDA): the form of will be discussed in Section 2.3. When an individual is treated this way they are prescribed radical cure with probability , otherwise they receive blood-stage treatment. An individual undergoes treatment for an average of days (14-day primaquine) at which point they may: die with probability , remain with asymptomatic blood-stage malaria with probability , if they are left with latent hypnozoites () with probability , otherwise they recover (). Similarly, an individual ends blood-stage treatment after an average of days (3-day ACT) at which point they may: die with probability , remain with asymptomatic blood-stage malaria with probability , if they are left with latent hypnozoites with probability , otherwise they recover. Latent stage P. vivax infected individuals experience a relapse at rate or they are reinfected at rate (where represents a possible reduction in susceptibility due to anti-parasite immunity) [24]. Upon relapse or reinfection from the individual experiences clinical malaria with probability (where ). Recovered individuals () are reinfected with rate at which point they become a clinical case with probability (where ). In addition to the possibility of relapse or reinfection, recovered and latent individuals lose immunity and hypnozoites at rates and , respectively, and return to being susceptible.
For individuals with mixed infections, there are several transitions in the model which depend on the individual’s state with respect to both species: we refer to these dependencies as species “interactions”. When an individual with a mixed infection is treated, they move from states in to a state in for both species (depending on the treatment); this is referred to as “treatment entanglement”. Similarly, when a patient stops treatment with respect to one malaria species, they are moved to one of the post-treatment states with respect to the other species. Death with respect to one species will cause a transition to death with respect to the other. The model allows treatment efficacies to vary for mixed infections; however, in this work we have assumed antimalarial efficacy against each species to be equivalent to the efficacy against mono-infections. We model P. vivax relapses triggered by the recovery of P. falciparum (“triggering”) by setting the relapse rate for a person that is recovered () from P. falciparum but has latent stage () P. vivax to be , where . The model also allows blood-stage P. vivax to be masked by blood-stage P. falciparum (“masking”) by treating a mixed infection as though it were a P. falciparum infection only with probability . Explicitly, for an individual with P. falciparum and P. vivax both in states or , the probability of receiving radical cure is .
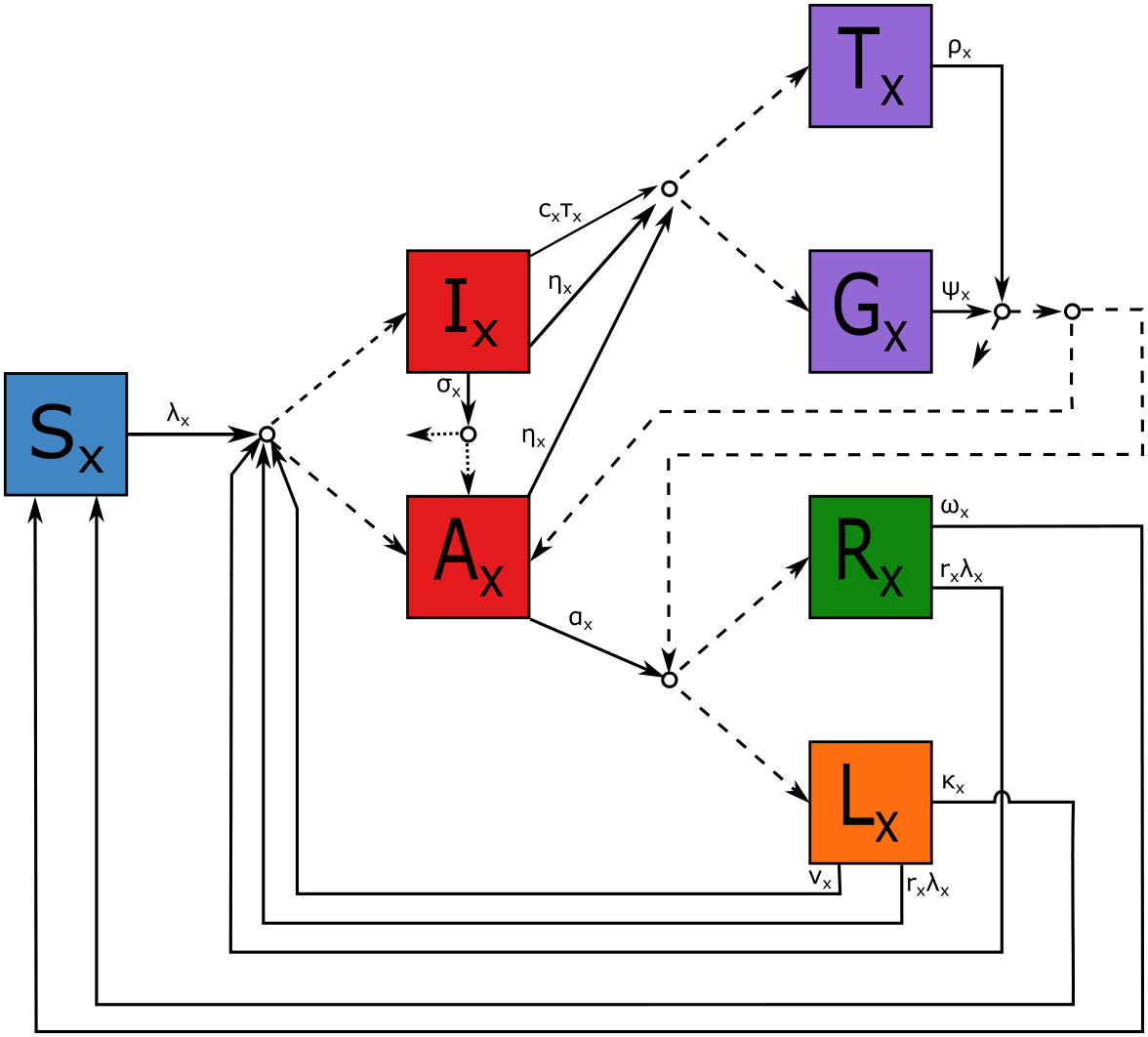
2.2 Transmission intensity and vector species
The dynamics of the mosquito population are governed by a system of ODEs (presented in the Supplementary §2). The mosquitoes follow standard Susceptible-Exposed-Infectious (SEI) dynamics with the addition of a seasonally varying death rate and the ability for mosquitoes to carry and spread mixed infection in a single bite (known as simultaneous inoculation). Asymptomatic individuals tend to have a lower peripheral parasitaemia and therefore were assumed to be less infectious to mosquitoes than individuals with clinical malaria with a relative infectiousness of .
We consider model parameters that would be representative of a Cambodia-like context, where both P. falciparum and P. vivax circulate, and the mosquito species present (An. dirus, An. minimus, An. maculatus, and An. barbirostris) are able to transmit both parasite species [25] allowing mosquitoes to be modelled as a single population that spreads both P. falciparum and P. vivax.
2.3 Treatment Scenarios
We simulate three treatment scenarios: current practice, accelerated radical cure, and unified radical cure. In each scenario we assume that when a person tests positive for malaria the species is always identified correctly for mono-infections, since specialised RDTs have been shown to have high sensitivity and specificity, particularly for P. falciparum (see, for example, [12]). The three treatment practices considered are:
-
1.
Current practice: Under this scenario, P. falciparum and most P. vivax cases are prescribed a blood-stage treatment but only 16% of P. vivax cases are prescribed radical cure. The low coverage of radical cure was chosen to match the rates reported from a study in Cambodia where radical cure was prescribed conservatively to 16% of detected P. vivax cases [26]. That is, the probability that an individual receives radical cure when being treated for malaria , is
where is the probability that P. vivax is masked by P. falciparum when the individual is tested.
-
2.
Accelerated radical cure: Under this scenario, any eligible person who is diagnosed with P. vivax and returns a G6PD normal RDT is prescribed radical cure with a low-dose 14-day course of primaquine (total dose 3.5 mg/kg) alongside a 3-day course of blood-stage treatment. Anyone who is 6 months old and is not pregnant or lactating is considered eligible for radical cure. We assume that the G6PD RDTs have a sensitivity of 94% and a specificity of 91% [27], 6% of the population have G6PD enzyme activity 30%, 2% of the population are pregnant and/or lactating and 2% of the population are 6 months old. When combined, this means that 18% of people diagnosed with P. vivax will be ineligible to receive radical cure (further details are given Supplementary §3). The probability of receiving radical cure under this scenario is
-
3.
Unified radical cure: Under this scenario, radical cure is prescribed to any eligible person in whom peripheral parasitaemia is detected, with eligibility as defined and calculated in the accelerated radical cure scenario. The probability of receiving radical cure in this scenario is
The three treatment scenarios and the probability of receiving radical cure is summarised in Table 1 with an assumed probability of masking of .
Current Practice | Accelerated RC | Unified RC | |
Treatments | |||
P. falciparum | ACT + PQ1 | ACT + PQ1 | ACT + PQ14 |
P. vivax | CQ + PQ14 | CQ + PQ14 | ACT + PQ14 |
Mixed | ACT + PQ14 | ACT + PQ14 | ACT + PQ14 |
Radical Cure Coverage (given detected infection) | |||
P. falciparum | 0 | 0 | 0.82 |
P. vivax | 0.16 | 0.82 | 0.82 |
Mixed | 0.08 | 0.41 | 0.82 |
For each of the three treatment scenarios we also consider the impact of an MDA intervention where a proportion of the population are given a blood-stage treatment irrespective of infective status, thus allowing a proportion of clinical and asymptomatic blood-stage infections to be treated. We assume that a proportion, , of the population are prescribed a blood-stage treatment over a fixed period of time, , so that the treatment rate of an individual with species due to MDA is:
We assume that people are not screened for G6PD status nor prescribed radical cure during MDA, based on concerns about haemolytic risks outweighing the benefits in patients who do not have malaria [28]. That is, the probability that an individual receives radical cure under MDA, given that they are treated for malaria type is = 0 for all .
2.4 Implementation
We present the impact of different treatment and intervention strategies on the number of malaria cases and deaths from 2021 to 2030, where 2030 is the regional target for malaria elimination.
For each treatment and intervention scenario we run 50 model simulations and record the model compartments over time. Given the relatively short time frame, we ignore background human demographic dynamics in our model to reduce computational complexity.
For the current practice scenarios we assume that radical cure is prescribed conservatively and does not increase the risk of haemolysis (as a best case scenario). To account for heamolytic risk in the accelerated radical cure and unified radical cure scenarios we increase the probability of death by for patients administered primaquine and the probability of radical cure failure by (details on these values are given in Supplementary §3).
For the MDA intervention, we assume that half of the population receive blood-stage treatment over a 30 day period. The MDA is rolled out twice yearly, before and after the annual peak in transmission.
Initial conditions are set to resemble an endemic setting in eastern Cambodia, because the prevalence of clinical and asymptomatic infections in the region is well documented [29] and levels of immunity in eastern Cambodia were studied in a 2005 sero-survey [30]. Some parameter values were based on expert elicitation or a limited evidence base, and some parameter estimates vary greatly between studies. As such, the scenarios presented here are indicative of population dynamics of multi-species infections over time that accommodates interaction between P. falciparum and P. vivax infections and expected trends in the impacts of different interventions. Accordingly, the scenarios here should not be interpreted as forecasts of malaria cases and deaths in Cambodia and other similar malaria-endemic regions over the next 10 years. All parameters and initial conditions are given in Supplementary Tables S2 and S3.
We performed a sensitivity analysis on the model, in which each model parameter was modified separately and the relative change in model outputs recorded. To implement the sensitivity analysis, we considered the baseline value of each parameter (given in Tables 2 and 3) and ran simulations with the parameter scaled down to 80% and up to 120% while all other parameters remained fixed. If scaling a probability parameter up to 120% compared to baseline led to a probability being greater than 1 the value was instead held at 1. Similarly, the relative susceptibility of partially-immune individuals compared to susceptible individuals was not scaled up, so as not to exceed 1. For each set of parameter values, 50 repeats of the simulation were conducted and various outputs were recorded, including: the total number of P. falciparum infections, P. vivax infections, clinical P. falciparum infections, clinical P. vivax infections, P. vivax relapses, deaths, blood-stage treatments administered and radical cure treatments administered.
3 Results
3.1 Scenario modelling
Figure 2 depicts the total number of infectious individuals in the population over time (the median, minimum and maximum of the 50 simulations) and figures showing all model compartments through time are presented in Supplementary Figures S2 and S3. The different treatment strategies had little effect on the prevalence of P. falciparum, with the unified treatment scenario resulting in a marginally lower prevalence of P. falciparum. For P. vivax increasing the coverage of radical cure has a large impact on the prevalence of P. vivax, which appears to be approaching elimination towards the end of the time period. Meanwhile, the MDA intervention greatly reduced the prevalence of P. falciparum but had less of an effect on P. vivax. No scenarios led to elimination of malaria over the ten year period by 2030, although elimination may have been achieved over a longer time-frame.
Figure 3 shows boxplots of the cumulative number of infections and deaths by species over the 10 year period. The unified treatment strategy with G6PD testing of all individuals resulted in fewer infections and fewer deaths due to P. falciparum and P. vivax, despite the increased risk of haemolysis from radical cure. The accelerated radical cure approach approximately halved P. vivax infections but resulted in a small increase in P. falciparum infections, this increase is likely due to a reduction in the prevalence of mixed infections, which are more likely to be detected than mono-infections, inadvertently causing a reduction in the detection rate of P. falciparum.
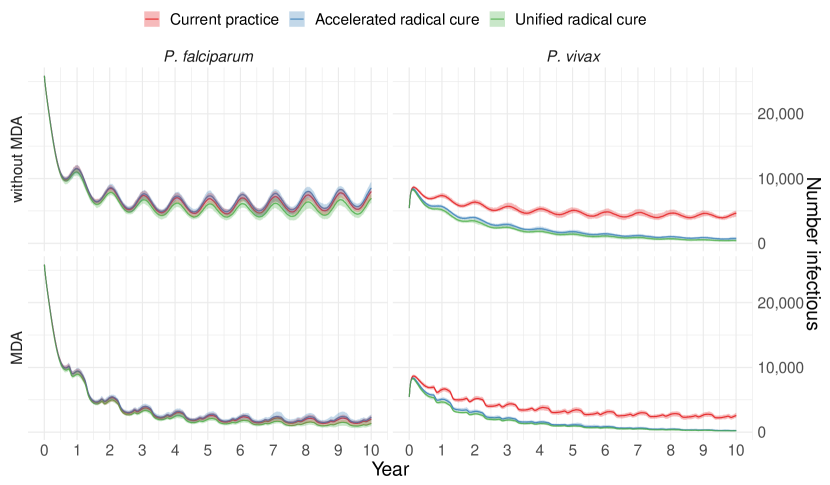
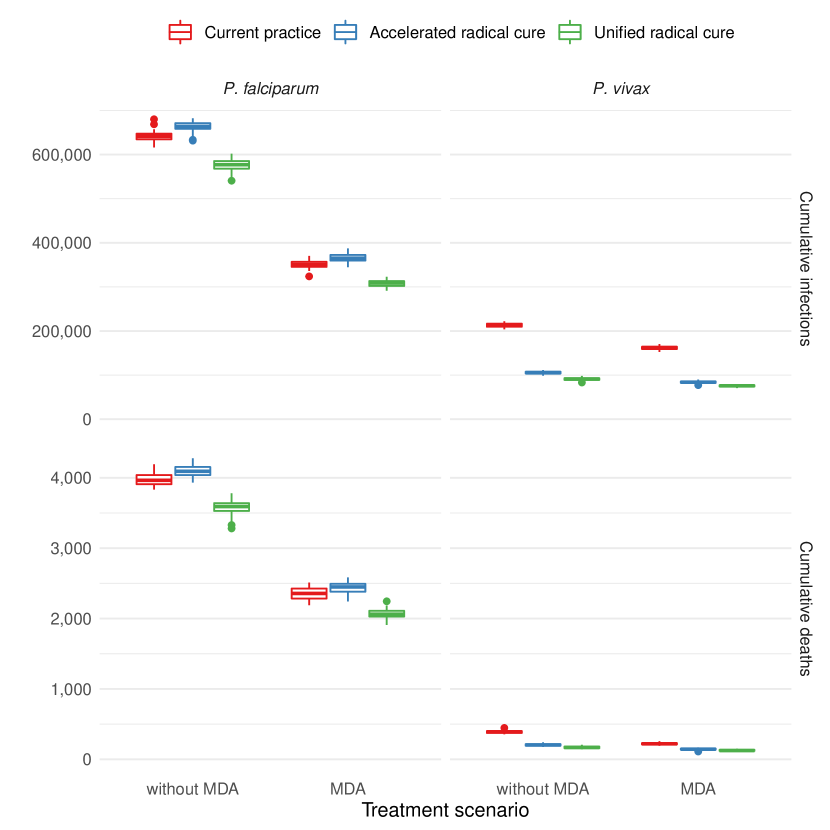
3.2 Sensitivity analysis
In Figure 4, the results of the sensitivity analysis are presented in terms of the ten most influential parameters on cumulative infections (including clinical and asymptomatic cases). Sensitivity analyses with respect to all parameters and other outcomes are given in Supplementary Figure S4. These figures present the mean, minimum and maximum relative outcome compared to the baseline over 50 simulations, for each parameter set and orders them based on their relative sensitivity (in terms of absolute difference between the 80% and 120% scenarios).
In Figure 4 we see that P. falciparum and P. vivax infections were most sensitive to many of the vector-related parameters, including: the bite rate (), the death rate of mosquitoes (), the probability of transmission given an infectious bite (from humans to mosquitoes and vice versa, and ) and the rate at which exposed mosquitoes become infectious (). The spread of mosquito-borne infectious diseases are well known to be sensitive to these parameters [31].
Aside from the vector-related parameters, we identified several important human-related parameters, including: the relative infectiousness of asymptomatic carriers (), the relative susceptibility of partially-immune individuals (), the rate at which asymptomatic infections are cleared (), the rate of treatment seeking () and accessibility of treatment (). The parameters and determine the expected number of secondary infections generated by asymptomatic individuals. The parameter is related to anti-parasite immunity, it represents a possible lower rate of infection in recovered individuals. The parameters and determine the rate at which clinical cases seek treatment and the probability that they receive treatment for malaria.
In addition to the parameters that were influential on cumulative infections of both species, P. vivax infections were sensitive to the probability that hypnozoites are cleared when blood-stage parasites are cleared without treatment (). This emphasises the contribution of relapses in the overall P. vivax malaria burden.
Other model outcomes (clinical infections, total deaths, total relapses, total treatments received) were broadly sensitive to the same model parameters without any additions or omissions (see Supplement Figure 4 for details). Small differences in relative sensitivity between measuring cumulative infections versus clinical infections were largely centred on the parameters having to do with the proportions immune expected to develop clinical malaria upon reinfection (that is, for P. falciparum and for P. vivax).
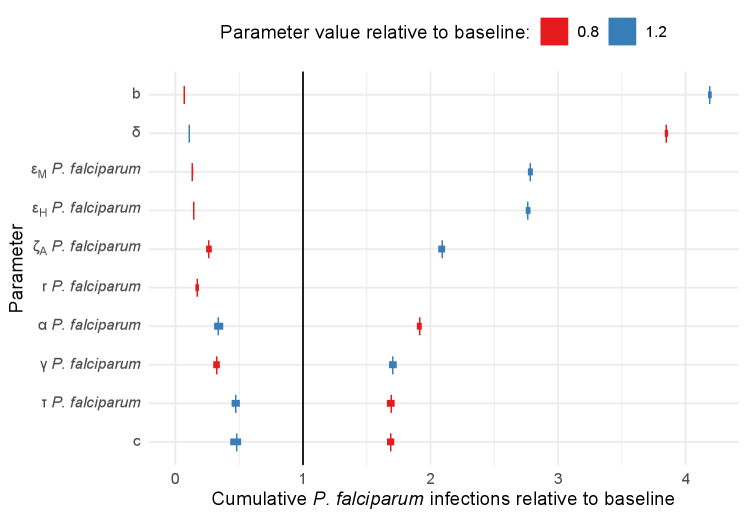
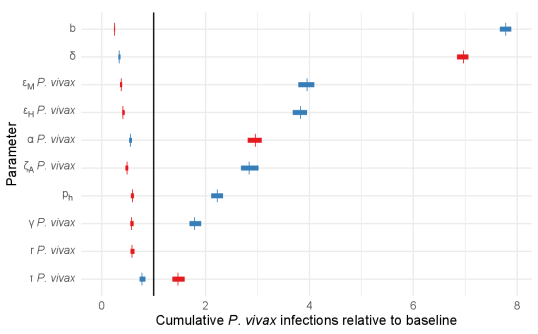
4 Discussion
Capturing the dynamics of multiple malaria species concurrently is policy-relevant but to date has drawn little attention. In this paper, we have developed a model of sufficient complexity to capture these dynamics, demonstrating how it can be used to inform health policy. Our model incorporates the dynamics of both P. falciparum and P. vivax in a way that captures masking, treatment entanglement and triggering interactions of the species. This is the second multi-species model which meaningfully captures dependencies between P. falciparum and P. vivax [23] and is the only stochastic, agent-based model to do so. The stochasticity in our approach makes it particularly well suited to model P. falciparum and P. vivax in low transmission settings, small populations, or as malaria is approaching elimination. The model also has fewer compartments, and fewer parameters, than the only other model with similar features [23], making our model more readily parameterised.
Our scenario analysis explored the effect of different proportions of coverage with radical cure treatment, assuming that individuals were screened for G6PD deficiency prior to treatment. The scenario analysis showed that a unified radical cure strategy can reduce the prevalence of malaria cases and deaths overall, even when accounting for the increased risk of death due to haemolysis under radical cure. Radical cure is effective because it blocks transmission, kills blood-stage parasites and kills dormant hypnozoites. A unified radical cure strategy avoids issues associated with masking when administering targeted treatment, allows for a consistent protocol for malaria treatment, and does not require the speciation of malaria prior to treatment.
Modelling a twice-yearly MDA intervention allowed us to assess the additional impact that could be achieved by treating asymptomatic infections as a means to reduce malaria burden. We found that MDA is an effective way to reduce prevalence, but it will not necessarily lead to elimination if coverage is too low. This is in line with a report from WHO, based on a systematic review of 270 literature reports, which states that for MDA to be effective, at least 80% of the population should be treated [32]. Achieving those levels of population coverage may be difficult due to issues with compliance. Due to safety concerns about treating individuals with G6PD deficiency with radical cure, only MDA with blood-stage treatment was considered. Consequently we found that MDA decreased P. falciparum prevalence but had less of an effect on P. vivax prevalence. Targeted interventions may allow radical cure to be administered en masse (such as focal screen and treat, or mass screen and treat) [32]. Our modelling framework easily allows these other kinds of interventions to be incorporated through the time-varying treatment function, .
The sensitivity analysis shows that, although the model has many parameters, the outputs are sensitive to relatively few parameters. The most sensitive parameters for both species were those related to vector-dynamics, the bite rates, transmission probabilities, mosquito death rate and the infectious period of mosquitoes, which are well known to be influential for mosquito-spread diseases [31]. Additionally, the relative infectiousness of asymptomatic individuals (), the rate at which asymptomatic infections are cleared (), the relative susceptibility of partially-immune individuals (), the rate of treatment seeking () and accessibility of treatment () were all found to be influential. Note that and determine the proportion of clinical infections that go untreated and become asymptomatic. Further, and determine the expected number of secondary infections generated by asymptomatic individuals. These sensitivities highlight how important asymptomatic individuals can be in driving malaria burden and the need for interventions that target asymptomatic infections, such as MDA (explored in this work) or better diagnostics that can detect infections with low level parasitaemia.
The number of P. vivax infections was sensitive to the probability of asymptomatic carriers naturally clearing hypnozoites, reinforcing the notion that relapses contribute significantly to malaria burden, as has been shown empirically in an analysis of 68,361 patients in Indonesia [33]. It is worth noting that since we performed a one-dimensional sensitivity analysis, the results should only be interpreted as the output sensitivity with respect to each parameter in isolation, and not interpreted as a full quantification of model output variation. A full probabilistic sensitivity analysis is appropriate for assessing output uncertainty, particularly if using the model to inform public health policy.
The simulations in our scenario analyses show behaviour comparable to Cambodia with parameters consistent with literature and expert elicitation. Many of the model parameters are location-specific such as the bite rate, relative infectiousness of asymptomatic carriers, probability of death from radical cure and the initial model state. Future work will develop a statistical framework for fitting this model, so that it can be applied in other endemic settings where parameters may differ substantially. The complexity of the multi-species model poses a challenge to jointly fitting all model parameters because of the high dimension of the parameter space and the run time, which was on the scale of minutes for a single simulation over 10 years. Optimised approximate Bayesian inference methods such as Bayesian Optimization for Likelihood-Free Inference (BOLFI) and Likelihood-Free Inference by Ratio Estimation (LFIRE) may provide solutions to both of these challenges [34, 35]. If the run time of the stochastic model becomes prohibitive for inference, as may be the case when applied to larger populations with high prevalence (where capturing small fluctuations in low numbers is less important), a deterministic or hybrid model equivalent could be applied instead.
This modelling framework provides the basis for future malaria modelling studies to evaluate the impact of integrated malaria control packages in settings where P. falciparum and P. vivax are co-endemic. In particular, parameters in the model can be adjusted to consider vector control measures and to evaluate other treatments, such as single-dose tafenoquine, high-dose 7-day primaquine and triple ACTs. The multi-species malaria model was developed in a way that enables economic analyses through the separation of different treatments and outcomes for individuals given their treatment. In the future, costs and quality of life metrics can be evaluated alongside the impact on cases and deaths. For example, this model could be used to identify under which circumstances a unified treatment for malaria would be cost-effective. Lastly, the modelling framework could be expanded to include other species of malaria, such as zoonotic P. knowlesi.
4.1 Role of the funding source
ACREME funded the salary of RIH, and contributed to the costs of data cleaning and organisation by PN and the CNM.
Supplementary Material
Code and other supplementary material are provided on GitHub at https://github.com/jnwalker2/multispecies-malaria-model.
Acknowledgements
This work is supported in part by the Australian Centre for Research Excellence in Malaria Elimination (ACREME), funded by the NHMRC (1134989). J.A. Simpson is funded by an Australian National Health and Medical Research Council of Australia (NHMRC) Investigator Grant (1196068). R. N Price is a Wellcome Trust Senior Fellow in Clinical Science (200909). J.M. McCaw’s research is supported by the ARC (DP170103076, DP210101920) and ACREME. J.A. Flegg’s research is supported by the ARC (DP200100747, FT210100034). A. Devine’s research is supported by National Health and Medical Research Council of Australia (NHMRC) (APP1132975). The contents of the published material are solely the responsibility of the individual authors and do not reflect the views of NHMRC. A. Devine and D.J. Price’s research are supported by DFAT.
References
- [1] WHO. World malaria report 2021. Technical report, World Health Organization, Geneva, 2021.
- [2] Roly D Gosling, Maxine Whittaker, Cara Smith Gueye, Nancy Fullman, Mario Baquilod, Rita Kusriastuti, and Richard GA Feachem. Malaria elimination gaining ground in the Asia Pacific. Malaria Journal, 11(1):1–3, 2012.
- [3] WHO. WHO guidelines for malaria 2022. World Health Organization, Geneva, 2022.
- [4] Kamala Thriemer, Benedikt Ley, Albino Bobogare, Lek Dysoley, Mohammad Shafiul Alam, Ayodhia P Pasaribu, Jetsumon Sattabongkot, Elodie Jambert, Gonzalo J Domingo, Robert Commons, et al. Challenges for achieving safe and effective radical cure of Plasmodium vivax: a round table discussion of the APMEN Vivax Working Group. Malaria journal, 16(1):1–9, 2017.
- [5] Enny Kenangalem, Jeanne Rini Poespoprodjo, Nicholas M. Douglas, Faustina Helena Burdam, Ketut Gdeumana, Ferry Chalfein, Prayoga, Franciscus Thio, Angela Devine, Jutta Marfurt, Govert Waramori, Shunmay Yeung, Rintis Noviyanti, Pasi Penttinen, Michael J. Bangs, Paulus Sugiarto, Julie A. Simpson, Yati Soenarto, Nicholas M. Anstey, and Ric N. Price. Malaria morbidity and mortality following introduction of a universal policy of artemisinin-based treatment for malaria in Papua, Indonesia: A longitudinal surveillance study. PLOS Medicine, 16(5):1–23, 2019.
- [6] Ric N Price, Robert J Commons, Katherine E Battle, Kamala Thriemer, and Kamini Mendis. Plasmodium vivax in the era of the shrinking P. falciparum map. Trends in parasitology, 36(6):560–570, 2020.
- [7] Verena I. Carrara, Khin Maung Lwin, Aung Pyae Phyo, Elizabeth Ashley, Jacher Wiladphaingern, Kanlaya Sriprawat, Marcus Rijken, Machteld Boel, Rose McGready, Stephane Proux, Cindy Chu, Pratap Singhasivanon, Nicholas White, and François Nosten. Malaria burden and artemisinin resistance in the mobile and migrant population on the Thai–Myanmar border, 1999–2011: An observational study. PLOS Medicine, 10(3):e1001398, 2013.
- [8] Adeshina I. Adekunle, Mykola Pinkevych, Rose McGready, Christine Luxemburger, Lisa J. White, François Nosten, Deborah Cromer, and Miles P. Davenport. Modeling the dynamics of Plasmodium vivax infection and hypnozoite reactivation in vivo. PLOS Neglected Tropical Diseases, 9(3):e0003595, 2015.
- [9] Nicholas M. Douglas, George K. John, Lorenz von Seidlein, Nicholas M. Anstey, and Ric N. Price. Chemotherapeutic strategies for reducing transmission of Plasmodium vivax malaria. Advances in Parasitology, 80:271–300, 2012.
- [10] Jordi Landier, Daniel M Parker, Aung Myint Thu, Khin Maung Lwin, Gilles Delmas, François H Nosten, Chiara Andolina, Ricardo Aguas, Saw Moe Ang, Ei Phyo Aung, Naw Baw Baw, Saw Aye Be, Saw B’Let, Hay Bluh, Craig A. Bonnington, Victor Chaumeau, Miasa Chirakiratinant, Win Cho Cho, Peter Christensen, Vincent Corbel, Nicholas PJ Day, Saw Hsa Dah, Gilles Delmas, Mehul Dhorda, Arjen M Dondorp, Jean Gaudart, Gornpan Gornsawun, Warat Haohankhunnatham, Saw Kyaw Hla, Saw Nay Hsel, Gay Nay Htoo, Saw Nay Htoo, Mallika Imwong, Saw John, Ladda Kajeechiwa, Lily Kereecharoen, Praphan Kittiphanakun, Keerati Kittitawee, Kamonchanok Konghahong, Saw Diamond Khin, Saw Win Kyaw, Jordi Landier, Clare Ling, Khin Maung Lwin, Khine Shwe War Lwin, Naw K’ Yin Ma, Alexandra Marie, Cynthia Maung, Ed Marta, Myo Chit Minh, Olivo Miotto, Paw Khu Moo, Ku Ler Moo, Merry Moo, Naw Na Na, Mar Nay, François H. Nosten, Suphak Nosten, Slight Naw Nyo, Eh Kalu Shwe Oh, Phu Thit Oo, Tun Pyit Oo, Daniel M. Parker, Eh Shee Paw, Choochai Phumiya, Aung Pyae Phyo, Kasiha Pilaseng, Stéphane Proux, Santisuk Rakthinthong, Wannee Ritwongsakul, Kloloi Salathibuphha, Armon Santirad, Sunisa Sawasdichai, Lorenz von Seidlein, Paw Wah Shee, Paw Bway Shee, Decha Tangseefa, Aung Myint Thu, May Myo Thwin, Saw Win Tun, Chode Wanachaloemlep, Lisa J White, Nicholas J White, Jacher Wiladphaingern, Saw Nyunt Win, Nan Lin Yee, and Daraporn Yuwapan. Effect of generalised access to early diagnosis and treatment and targeted mass drug administration on Plasmodium falciparum malaria in Eastern Myanmar: an observational study of a regional elimination programme. The Lancet, 391(10133):1916–1926, 2018.
- [11] Srean Chhim, Patrice Piola, Tambri Housen, Vincent Herbreteau, and Bunkea Tol. Malaria in Cambodia: A retrospective analysis of a changing epidemiology 2006–2019. International Journal of Environmental Research and Public Health, 18(4), 2021.
- [12] Katharine Abba, Amanda J Kirkham, Piero L Olliaro, Jonathan J Deeks, Sarah Donegan, Paul Garner, and Yemisi Takwoingi. Rapid diagnostic tests for diagnosing uncomplicated non-falciparum or Plasmodium vivax malaria in endemic countries. Cochrane Database Syst Rev, 2014(12):CD011431, 2014.
- [13] Ruth A. Ashton, Takele Kefyalew, Gezahegn Tesfaye, Helen Counihan, Damtew Yadeta, Bonnie Cundill, Richard Reithinger, and Jan H. Kolaczinski. Performance of three multi-species rapid diagnostic tests for diagnosis of Plasmodium falciparum and Plasmodium vivax malaria in Oromia Regional State, Ethiopia. Malaria Journal, 9(1):297, 2010.
- [14] Robert J. Commons, Julie A. Simpson, Kamala Thriemer, Mohammad S. Hossain, Nicholas M. Douglas, Georgina S. Humphreys, Carol H. Sibley, Philippe J. Guerin, and Ric N. Price. Risk of Plasmodium vivax parasitaemia after Plasmodium falciparum infection: a systematic review and meta-analysis. The Lancet. Infectious Diseases, 19(1):91–101, 2019.
- [15] Jessica T. Lin, Delia Bethell, Stuart D. Tyner, Chanthap Lon, Naman K. Shah, David L. Saunders, Sabaithip Sriwichai, Phisit Khemawoot, Worachet Kuntawunggin, Bryan L. Smith, Harald Noedl, Kurt Schaecher, Duong Socheat, Youry Se, Steven R. Meshnick, and Mark M. Fukuda. Plasmodium falciparum gametocyte carriage is associated with subsequent Plasmodium vivax relapse after treatment. PLOS ONE, 6(4):1–8, 04 2011.
- [16] Mohammad S. Hossain, Robert J. Commons, Nicholas M. Douglas, Kamala Thriemer, Bereket H. Alemayehu, Chanaki Amaratunga, Anupkumar R. Anvikar, Elizabeth A. Ashley, Puji B. S. Asih, Verena I. Carrara, Chanthap Lon, Umberto D’Alessandro, Timothy M. E. Davis, Arjen M. Dondorp, Michael D. Edstein, Rick M. Fairhurst, Marcelo U. Ferreira, Jimee Hwang, Bart Janssens, Harin Karunajeewa, Jean R. Kiechel, Simone Ladeia-Andrade, Moses Laman, Mayfong Mayxay, Rose McGready, Brioni R. Moore, Ivo Mueller, Paul N. Newton, Nguyen T. Thuy-Nhien, Harald Noedl, Francois Nosten, Aung P. Phyo, Jeanne R. Poespoprodjo, David L. Saunders, Frank Smithuis, Michele D. Spring, Kasia Stepniewska, Seila Suon, Yupin Suputtamongkol, Din Syafruddin, Hien T. Tran, Neena Valecha, Michel Van Herp, Michele Van Vugt, Nicholas J. White, Philippe J. Guerin, Julie A. Simpson, and Ric N. Price. The risk of Plasmodium vivax parasitaemia after P. falciparum malaria: An individual patient data meta-analysis from the Worldwide Antimalarial Resistance Network. PLOS Medicine, 17(11):1–26, 2020.
- [17] Jeanne Rini Poespoprodjo, Faustina Helena Burdam, Freis Candrawati, Benedikt Ley, Niamh Meagher, Enny Kenangalem, Ratni Indrawanti, Leily Trianty, Kamala Thriemer, David J Price, et al. Supervised versus unsupervised primaquine radical cure for the treatment of falciparum and vivax malaria in Papua, Indonesia: a cluster-randomised, controlled, open-label superiority trial. The Lancet Infectious Diseases, 22(3):367–376, 2022.
- [18] Sandip Mandal, Ram Sarkar, and Somdatta Sinha. Mathematical models of malaria - a review. Malaria Journal, 10(1):202, 2011.
- [19] Neal R. Smith, James M. Trauer, Manoj Gambhir, Jack S. Richards, Richard J. Maude, Jonathan M. Keith, and Jennifer A. Flegg. Agent-based models of malaria transmission: a systematic review. Malaria Journal, 17(1):299, 2018.
- [20] Ricardo Aguas, Marcelo U. Ferreira, and M. Gabriela M. Gomes. Modeling the effects of relapse in the transmission dynamics of malaria parasites. Journal of Parasitology Research, 2012, 2012.
- [21] Puntani Pongsumpun and I-Ming Tang. Mathematical model for the transmission of P. falciparum and P. vivax malaria along the Thai-Myanmar border. Int J Biol Life Sci, 3, 2008.
- [22] Puntani Pongsumpun and I-Ming Tang. Impact of cross-border migration on disease epidemics: case of the P. falciparum and P. vivax malaria epidemic along the Thai-Myanmar border. Journal of Biological Systems, 18(01):55–73, 2010.
- [23] Rima Shretta, Sheetal Prakash Silal, Olivier J. Celhay, Chris Erwin Gran Mercado, Shwe Sin Kyaw, Anton Avancena, Katie Fox, Brittany Zelman, Ranju Baral, Lisa Jane White, and Richard James Maude. Malaria elimination transmission and costing in the Asia-Pacific: Developing an investment case. Wellcome Open Research, 4(60), 2020.
- [24] Denise L. Doolan, Carlota Dobaño, and J. Kevin Baird. Acquired immunity to malaria. Clinical Microbiology Reviews, 22(1):13–36, 2009.
- [25] Sovannaroth Siv, Arantxa Roca-Feltrer, Seshu Babu Vinjamuri, Denis Mey Bouth, Dysoley Lek, Mohammad Abdur Rashid, Ngau Peng By, Jean Popovici, Rekol Huy, and Didier Menard. Plasmodium vivax malaria in Cambodia. The American Journal of Tropical Medicine and Hygiene, 95(6):97–107, 2016.
- [26] Stefan Hoyer, Sokomar Nguon, Saorin Kim, Najibullah Habib, Nimol Khim, Sarorn Sum, Eva-Maria Christophel, Steven Bjorge, Andrew Thomson, Sim Kheng, Nguon Chea, Sovann Yok, Samphornarann Top, Seyha Ros, Uth Sophal, Michelle M. Thompson, Steve Mellor, Frédéric Ariey, Benoit Witkowski, Chhiang Yeang, Shunmay Yeung, Socheat Duong, Robert D. Newman, and Didier Menard. Focused screening and treatment (FSAT): A PCR-based strategy to detect malaria parasite carriers and contain drug resistant P. falciparum, Pailin, Cambodia. PLOS ONE, 7(10):1–12, 2012.
- [27] Benedikt Ley, Ari Winasti Satyagraha, Hisni Rahmat, Michael E. von Fricken, Nicholas M. Douglas, Daniel A. Pfeffer, Fe Espino, Lorenz von Seidlein, Gisela Henriques, Nwe Nwe Oo, Didier Menard, Sunil Parikh, Germana Bancone, Amalia Karahalios, and Ric N. Price. Performance of the Access Bio/CareStart rapid diagnostic test for the detection of glucose-6-phosphate dehydrogenase deficiency: A systematic review and meta-analysis. PLOS Medicine, 16:1–15, 2019.
- [28] Ken Ing Cherng Ong, Hodaka Kosugi, Sophea Thoeun, Hitomi Araki, Moe Moe Thandar, Moritoshi Iwagami, Bouasy Hongvanthong, Paul T Brey, Shigeyuki Kano, and Masamine Jimba. Systematic review of the clinical manifestations of glucose-6-phosphate dehydrogenase deficiency in the Greater Mekong Subregion: implications for malaria elimination and beyond. BMJ global health, 2(3):e000415, 2017.
- [29] Mirco Sandfort, Amélie Vantaux, Saorin Kim, Thomas Obadia, Anaïs Pepey, Soazic Gardais, Nimol Khim, Dysoley Lek, Michael White, Leanne J. Robinson, Benoit Witkowski, and Ivo Mueller. Forest malaria in Cambodia: the occupational and spatial clustering of Plasmodium vivax and Plasmodium falciparum infection risk in a cross-sectional survey in Mondulkiri province, Cambodia. Malaria Journal, 19(1):413, 2020.
- [30] Jackie Cook, Nico Speybroeck, Tho Sochanta, Heng Somony, Mao Sokny, Filip Claes, Kristel Lemmens, Michael Theisen, Irene S. Soares, Umberto D’Alessandro, Marc Coosemans, and Annette Erhart. Sero-epidemiological evaluation of changes in Plasmodium falciparum and Plasmodium vivax transmission patterns over the rainy season in Cambodia. Malaria Journal, 11(1):86, 2012.
- [31] Nakul Chitnis, James M. Hyman, and Jim M. Cushing. Determining important parameters in the spread of malaria through the sensitivity analysis of a mathematical model. Bulletin of Mathematical Biology, 70(5):1272, 2008.
- [32] WHO. Meeting report of the Evidence Review Group on mass drug administration, mass screening and treatment and focal screening and treatment for malaria. World Health Organization, Geneva, 2015.
- [33] Saber Dini, Nicholas M Douglas, Jeanne Rini Poespoprodjo, Enny Kenangalem, Paulus Sugiarto, Ian D Plumb, Ric N Price, and Julie A Simpson. The risk of morbidity and mortality following recurrent malaria in Papua, Indonesia: a retrospective cohort study. BMC medicine, 18(1):1–12, 2020.
- [34] Michael U. Gutmann, Jukka Cor, and er. Bayesian optimization for likelihood-free inference of simulator-based statistical models. Journal of Machine Learning Research, 17(125):1–47, 2016.
- [35] Owen Thomas, Ritabrata Dutta, Jukka Corander, Samuel Kaski, and Michael U. Gutmann. Likelihood-free inference by ratio estimation. Bayesian Analysis, 17(1):1 – 31, 2022.