Emergence of 6-particle “hexciton” states in WS2 and MoSe2 monolayers
Abstract
When doped with a high density of mobile charge carriers, monolayer transition-metal dichalcogenide (TMD) semiconductors can host new types of composite many-particle exciton states that do not exist in conventional semiconductors. Such multi-particle bound states arise when a photoexcited electron-hole pair couples to not just a single Fermi sea that is quantum-mechanically distinguishable (as for the case of conventional charged excitons or trions), but rather couples simultaneously to multiple Fermi seas, each having distinct spin and valley quantum numbers. Composite six-particle “hexciton” states were recently identified in electron-doped WSe2 monolayers, but under suitable conditions they should also form in all other members of the monolayer TMD family. Here we present spectroscopic evidence demonstrating the emergence of many-body hexcitons in charge-tunable WS2 monolayers (at the A-exciton) and MoSe2 monolayers (at the B-exciton). The roles of distinguishability and carrier screening on the stability of hexcitons are discussed.
The monolayer transition-metal dichalcogenide (TMD) semiconductors such as WSe2, MoSe2, WS2, and MoS2 host a multitude of excitonic complexes, due in part to the spin-orbit-split nature of their conduction and valence bands at the and valleys of the Brillouin zone Urbaszek:2018 ; Muller:2018 . When they are optically allowed and possess a non-zero oscillator strength, these bound complexes manifest as discrete resonances in optical absorption spectra. Early studies of nominally undoped TMD monolayers revealed pronounced absorption peaks from the fundamental electron-hole optical transition (i.e., the neutral exciton) Splendiani:2010 ; Mak:2010 ; Li:2014 . Subsequent studies of charge-tunable TMD monolayers demonstrated the emergence, at lower energy, of additional strong absorption lines when the monolayer was populated with a background Fermi sea of holes or electrons (i.e., the charged excitons) Ross:2013 ; Mak:2013 ; Wang:2017NL . Whether, and under what conditions, a complex is most accurately described as a simple three-particle ‘trion’ (wherein the photoexcited exciton binds a carrier from the Fermi sea Ross:2013 ; Mak:2013 ; Wang:2017NL ; Kheng:1993 ; Astakhov:2002 ; BarJoseph:2005 ), or a four-particle ‘tetron’ (a trion additionally correlated with the resulting hole that is left behind in the Fermi sea Bronold:2000 ; Suris:2001 ; Suris:2003 ; Combescot:2018 ; Rana:2020 ), or an ‘exciton-polaron’ (an exciton dressed by collective excitations of the Fermi sea Efimkin:2017 ; Sidler:2016 ), remains an active area of study and discussion Reichman:2019 ; Glazov:2020 ; Rana:2021 ; Efimkin:2021 ; Fey:2020 ; Liu:2021 .
Regardless of interpretation, all of these descriptions share a common understanding: are bound states arising from the interaction of a photoexcited electron-hole (e-h) pair with the subset of carriers in the Fermi sea that have distinguishable quantum numbers. In most conventional III-V and II-IV semiconductors such as GaAs or ZnSe, where band extrema occur at the single central -point valley of the Brillouin zone Kheng:1993 ; Astakhov:2002 ; BarJoseph:2005 , this means that forms with mobile carriers having opposite spin to that of the photoexcited electron or hole because Pauli exclusion prevents strong short-range interactions with same-spin carriers. However, the multi-valley nature of monolayer TMDs expands the basis set of available quantum numbers, and band-edge electrons and holes can be distinguished not only by their spin (up or down) but also by their valley degree of freedom ( or ). TMD monolayers therefore permit, under suitable conditions, photoexcited e-h pairs to interact with Fermi seas containing more than one type of quantum-mechanically distinguishable carrier. As demonstrated recently Li:2022 ; vanTuanPRL:2022 ; vanTuan:2022 , this leads to qualitatively new types of multi-particle composite exciton ground states that can be described as bound six-particle “hexcitons” (when photoexcited e-h pairs interact with two distinguishable Fermi seas) or even eight-particle “oxcitons” (when interacting with three distinguishable Fermi seas). These bound many-body excitonic states have large oscillator strengths and manifest as discrete absorption resonances in linear optical spectroscopy, and emerge at energies even further below and . We emphasize that these composite hexcitons are optically-allowed ground states of the interacting exciton-Fermi sea system, and are therefore distinct from the many types of optically-forbidden dark excitons and trions that appear only in photoluminescence studies Urbaszek:2018 ; Muller:2018 ; Robert:2017 ; Malic:2018 ; He:2020 ; Yang:2022 , and moreover should not be confused with multi-exciton complexes (such as biexcitons) that appear only at higher photoexcitation intensity Urbaszek:2018 ; Muller:2018 ; Barbone:2018 ; ZLi:2018 ; Chen:2018 .
To date, such multi-particle hexcitons have been identified and studied only in electron-doped WSe2 monolayers Li:2022 ; vanTuanPRL:2022 ; vanTuan:2022 . This is due to i) the excellent optical quality of exfoliated WSe2, ii) the ability to electrostatically dope WSe2 to high electron densities, and iii) because composite hexcitons in WSe2 are expected at the low-energy A-exciton optical transitions where spectral linewidths are typically much sharper than at the higher-energy B-exciton. This latter fact arises from the positive sign of the conduction band (CB) spin-orbit splitting in WSe2 () Song:2013 ; Kormanyos:2015 , which mandates that A-exciton optical transitions photoexcite electrons to the upper CBs in and , where Pauli exclusion does not prevent them from interacting with both of the distinguishable Fermi seas of electrons that occupy the two lower CBs (as depicted in the band diagram in Fig. 1, one Fermi sea resides in the same valley but has opposite spin, the other has same spin but resides in the opposite valley).
However, under appropriate conditions, composite hexcitons should also emerge in all members of the monolayer TMD family. For example, monolayer WS2 also has a positive and CB structure similar to WSe2, and therefore hexcitons should also appear at its A-exciton under conditions of high electron doping. In contrast, the negative of monolayer MoSe2 Song:2013 ; Kormanyos:2015 precludes the existence of hexcitons at its A-exciton (instead, only conventional should appear), but does allow for the formation of hexcitons at its B-exciton transition. To date, neither of these predictions have been explicitly tested. Here, using low-temperature optical absorption measurements of electrostatically-gated WS2 and MoSe2 monolayers, we demonstrate and investigate the emergence of composite hexcitons in both WS2 monolayers (at the A-exciton) and MoSe2 monolayers (at the B-exciton). Based on the data, the roles of distinguishability and carrier screening on the stability of hexcitons are discussed.
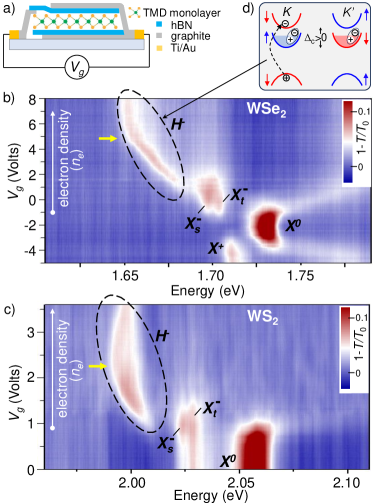
Figure 1a depicts the charge-tunable TMD monolayer samples studied in this work. Single monolayer flakes of WS2, MoSe2, and WSe2 were mechanically exfoliated and sandwiched between thin slabs of hexagonal boron nitride (hBN). Few-layer graphite flakes were used to electrically contact the monolayer, and to serve as top and bottom gates. In this work, the top and bottom gates were tied together and gate voltage was used to electrostatically dope the monolayers with a background Fermi sea of electrons or holes. Dual gating allows us to attain high carrier densities approaching 1013/cm2. Each assembled structure was then positioned and placed directly over the core of a single-mode optical fiber, to ensure a rigid and robust alignment between the optical path and the doped TMD monolayer. This experimental approach Li:2020 ; Stier:2018 mitigates the drift and vibration that can otherwise complicate optical studies of TMD monolayers at low temperatures and in the pulsed magnetic fields used in this work.
The sample-on-fiber assembly was then mounted on a purpose-built probe and loaded into a liquid helium cryostat. Broadband white light from a Xenon lamp was directed down the single mode fiber. Following transmission through the sample the light passed through a thin-film circular polarizer and was then retro-reflected and directed back into a multimode collection fiber. The transmitted light was dispersed in a 300 mm spectrometer and detected by a charge-coupled device (CCD). In this way the optical absorption from the doped TMD monolayer was directly measured as , where is the spectrum of the transmitted light and is a reference spectrum. We note that absorption spectra typically permit a straightforward evaluation and visualization of exciton oscillator strengths, in comparison to reflectivity studies where lineshapes depend sensitively on interference effects from the surrounding layer structure.
Figure 1b shows a map of the gate-dependent absorption spectra from a WSe2 monolayer at low temperature (4K) and at zero magnetic field, in the spectral range of its A-exciton. When V, the monolayer is at its charge neutrality point and only the neutral exciton () absorption resonance is observed. At increasingly negative or positive , the monolayer becomes lightly doped with mobile holes or electrons, and the well-known resonances appear at energies 20-35 meV below . As extensively described in previous works, are optically-active bound states arising from the interaction of the photoexcited e-h pair with the carriers in the Fermi sea that possess distinguishable quantum numbers from those of the photoexcited e-h pair (i.e., different spin and/or valley). Thus, only a single resonance appears on the hole-doped side, but two conventional resonances appear on the electron-doped side (the so-called singlet and triplet charged excitons) because there are two distinct Fermi seas with which to interact. The different energies of and stem from the different amplitude of the short-range electron-hole exchange interaction Glazov:2020 ; Hichri:2020 ; Courtade:2017 .
Most importantly, at higher the and resonances disappear and a new strong absorption resonance appears at even lower energy (15 meV below ), indicating the emergence of a new bound excitonic ground state with large oscillator strength. First observed in gated WSe2 monolayers in 2013 Jones:2013 and very clearly resolved in several subsequent studies Wang:2017NL ; Wang:2017 ; Barbone:2018 ; SuFeiShi:2020 ; Liu:2021 , this low-energy absorption resonance – occasionally called in earlier literature – completely dominates the absorption spectrum of WSe2 at high . Moreover, it does not appear in hole-doped WSe2 at the A-exciton, and does not appear in the A-exciton absorption spectrum of gated MoSe2 monolayers Wang:2017NL ; Smolenski:2019 . Recently, this absorption resonance was identified as a qualitatively new type of many-body composite (six-particle hexciton) state, arising from the simultaneous interaction of the photoexcited e-h pair with both of the Fermi seas that reside in the lower CBs of monolayer WSe2 Li:2022 ; vanTuanPRL:2022 ; vanTuan:2022 . As depicted in Fig. 1d, each of these two Fermi seas has quantum numbers that are distinguishable from those of the photoexcited electron (one has opposite spin, the other has opposite valley), and – extending the picture of being four-particle tetrons Suris:2003 ; Rana:2020 – the hexciton bound state comprises the photoexcited e-h pair, an electron from each of the two distinguishable Fermi seas, and the two Fermi holes that are left behind in the Fermi seas. As described recently, hexcitons are the stable ground state of this interacting exciton-Fermi sea system, and the Fermi holes not only ensure overall charge neutrality but also provide the ‘glue’ that binds the complex vanTuanPRL:2022 ; vanTuan:2022 .
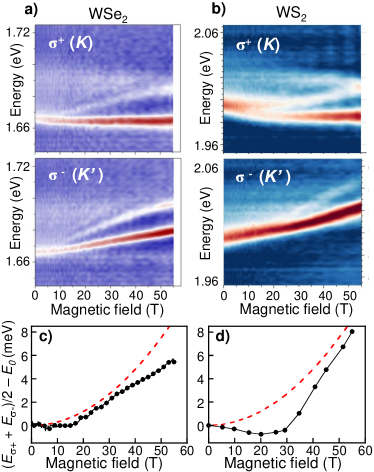
The ordering of the spin and valley polarized CBs in monolayer WS2 is similar to that of WSe2 (i.e., is also positive), and optical transitions at the A-exciton couple to the upper CBs. Consequently, optical signatures of composite hexcitons can therefore be anticipated at high in WS2 monolayers. The gate-dependent absorption map of Fig. 1c confirms this prediction: Strong absorption from is plainly visible at , and charged excitons appear at low . These conventional resonances in WS2 have been clearly resolved in several recent studies Kapuscinski:2020 ; Zipfel:2020 ; Zinkiewicz:2021 ; Robert:2021NC . Most importantly, our dual-gated structure allows a smooth tuning to a regime of high , where Fig. 1c shows that disappear and a new strong absorption resonance emerges at even lower energy (15 meV below ). The gate-dependent absorption of monolayer WS2 is therefore qualitatively identical to that of WSe2, albeit with broader linewidths that are likely due to the reduced material quality of sulfur-based TMDs. Thus, we associate the emergence of the low-energy absorption resonance with the stable formation of 6-particle hexciton states. Note that we were unable to dope our WS2 monolayers with mobile holes; even at large negative , only the neutral exciton was visible, likely due to strong mid-gap pinning of the Fermi level by the larger number of defects in sulfur-based TMDs.
Additional evidence supporting a picture of hexcitons in monolayer WS2 is the evolution of its optical resonance in applied magnetic fields . Figure 2 shows circularly polarized magneto-absorption from both WSe2 and WS2, under conditions of large where the hexciton absorption dominates. As shown recently Li:2022 , the hexciton resonance in WSe2 monolayers splits and shifts with increasing , and additional absorption resonances appear at higher energy that disperse linearly with and are related to the development of Landau levels (LLs) in the conduction and valence bands. Figure 2 shows that a qualitatively similar -dependent evolution of the hexciton peak also exists in WS2, where additional LL-like absorption features emerge for T. From the separation of these peaks we can estimate a combined electron and hole cyclotron resonance energy of 0.45 meV/T, which is slightly larger than obtained from WSe2 Li:2022 but in line with expectation given the slightly lighter carrier masses in WS2 Goryca:2019 .
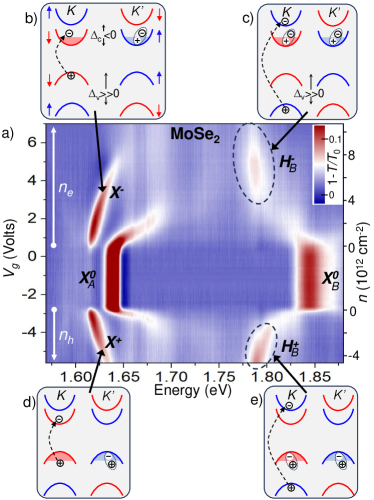
Moreover, from these spectra we can extract the diamagnetic shifts of the hexciton resonance, given by the average of the and absorption energies. This analysis, shown in Figs. 2c and 2d, reveals that the diamagnetic shifts of hexcitons – even at low – deviate from the purely quadratic behavior that is known to exist for neutral excitons in WSe2 and WS2 Stier:2018 ; Goryca:2019 (especially for WS2, where atypical diamagnetic shifts have also been observed for charged excitons Plechinger:2016 ). This behavior may arise from non-zero angular momentum contributions from the particles in the hexciton complex, and will be further investigated in future studies. For reference, however, the dotted red curves depict purely quadratic shifts () using a large diamagnetic coefficient eV/T2, which is larger than the known diamagnetic shifts of the very small and tightly-bound neutral excitons Stier:2018 ; Goryca:2019 . To the extent that these curves approximately capture the overall shifts of the hexciton resonances, these data are qualitatively consistent with recent calculations indicating that composite hexcitons are several times larger in size than neutral excitons vanTuanPRL:2022 ; vanTuan:2022
Taken together, these data provide evidence for the emergence of composite hexciton states in electron-doped monolayer WS2, which appear at the A-exciton owing to the positive sign of and consequent ordering of the spin-orbit-split CBs in the and valleys.
In marked contrast, neither electron-doped nor hole-doped MoSe2 monolayers show any indication of hexciton formation at the A-exciton, as shown in Fig. 3a. Rather – and as also observed in earlier studies Wang:2017NL ; Smolenski:2019 ; Liu:2021 – charge-tunable MoSe2 monolayers exhibit only a single and absorption that appears 25 meV below the neutral exciton . This observation is consistent with the negative sign of in MoSe2 Song:2013 ; Kormanyos:2015 , which dictates that optical transitions at the A-exciton photoexcite electrons to the lower (not upper) CBs. As such, when mobile electrons populate the lower CBs, only a single type of distinguishable electron exists in the Fermi sea, and many-body hexcitons cannot form (see diagrams in Fig. 3b,d).
The ordering of the CBs in MoSe2 does, however, permit hexciton formation at the higher energy B-exciton, where optical transitions couple to the upper CBs (see Fig. 3c). As Fig. 3a shows, when is small, the neutral B-exciton () at 1.84 eV disappears and only a faint and diffuse absorption remains at energies where conventional charged excitons are expected (that is, at about 25 meV below , or 1.815 eV). However, at larger ( cm-2), a stronger absorption with increased oscillator strength clearly emerges at 1.795 eV. Its separation from is 45 meV, which is larger than the 25 meV expected for conventional charged excitons, but is commensurate with the larger energy separation between hexcitons and neutral excitons that we observed in WSe2 and WS2 (cf. Fig. 1). Notably, Fig. 3 shows that this new absorption does not emerge until a large comparable to where the conventional trion loses oscillator strength, suggesting that it is not a conventional trion. Therefore, although past studies of charge-tunable MoSe2 monolayers have associated similar spectral signatures with conventional trions/tetrons/exciton-polarons of the B-exciton Wang:2017NL ; Liu:2021 , we argue that the low energy of the emerging absorption resonance and (especially) its dependence on are, in fact, more consistent with the formation and emergence of many-body hexcitons in electron-doped MoSe2 monolayers.
Furthermore, analogous signatures of hexcitons appear when the MoSe2 monolayer is doped with high concentrations of mobile holes, (see Fig. 3a). As depicted in the diagram of Fig. 3e, e-h pairs excited at the B-exciton will always have two distinguishable Fermi seas of mobile holes with which to interact, and composite hexcitons can be anticipated. Figure 3a shows that as starts to increase, both and disappear, and a conventional resonance appears 25 meV below when V. However, the concomitant response at the B-exciton is much weaker, until increases up to V, at which point a strong absorption emerges at a much lower energy of 40 meV below . This new resonance actually gains oscillator strength with increasing , while in this same doping range the conventional fades away. Based on this dependence, we rule out the possibility that the new resonance could be, e.g., a conventional trion associated with or an excited Rydberg state of . Moreover, this new resonance redshifts with increasing , similar to the redshift observed for hexcitons in electron-doped WSe2 and WS2 (cf. Fig. 1). These data therefore support a picture of robust hexciton formation at the B-exciton in hole-doped MoSe2. The resonance features are rather broad, however, likely due to the shorter lifetime of B-excitons.
Indeed, owing to the large 100s-of-meV spin-orbit splitting of the valence bands () that exists in all monolayer TMD semiconductors, robust hexciton formation at the B-exciton should emerge when any TMD monolayer semiconductor is doped with a high density of mobile holes (see diagram in Fig. 3e). Furthermore, because is large, the Fermi sea of holes never occupies the valence bands from which the photoexcited e-h pair originates, and the photohole always remains distinguishable from every hole in the Fermi sea. Hexcitons under such conditions should therefore remain robust and should redshift due to the primary effects of bandgap renormalization Scharf:2019 up to very large values of . While in this work we have studied B-excitons only in MoSe2, we note that the seminal work of Wang et al. Wang:2017NL clearly revealed a strong absorption below in heavily hole-doped WSe2 monolayers, that redshifted with increasing and did not broaden, consistent with hexciton formation.
An important insight gained from these various results is that the decay and broadening of excitonic complexes is likely governed more by the quantum-mechanical distinguishability of the photoexcited e-h pair (in relation to the carriers in the Fermi sea), than by screening from the Fermi sea. For example, in the case of electron-doped WSe2 and WS2 shown in Fig. 1, the redshifts of the hexciton resonances in WSe2 and WS2 cease, and their decay/broadening begins, only when electrons begin to populate the upper CBs. Beyond this point, the photoexcited electron is no longer distinguishable from every electron in the Fermi sea, and the Pauli exclusion principle dictates that when it is introduced into the (now occupied) upper CB, it must scatter away those mobile electrons having similar spin and valley quantum numbers vanTuan:2022 . This exchange scattering process leads to the decay and broadening of the hexciton resonance. Hexciton resonances therefore retain their amplitude and narrow linewidth when the charge density increases, as long as the photoexcited e-h pair remains distinguishable from all carriers in the Fermi sea. In hole-doped TMD monolayers, the large ensures distinguishability of e-h pairs excited at the B-exciton, and therefore a robust stability of hexcitons even to very large .
Moreover, screening by mobile carriers does not seem to be the primary driving force for broadening of the hexciton resonance, as inferred by the observation that the conventional resonances begin to lose oscillator strength at much smaller carrier density. For example, Fig. 3 shows that the conventional trions of MoSe2 around 1.63 eV decay when V. Concomitantly, however, the type-B hexciton in hole-doped conditions around 1.8 eV neither broadens nor decays at the same (and even higher) . A similar behavior was also measured for a wider range by Liu et al. Liu:2021 , where the type-B resonance around 1.8 eV maintains a large oscillator strength and continues to redshift long after the resonance at the A-exciton decays. This suggests that screening is not the primary cause of decay and broadening, because the Coulomb potential cannot selectively weaken the attraction between particles of one complex species but not of another.
In summary, composite hexcitons are expected in all members of the monolayer TMD family. When doped with a high density of electrons, hexcitons can emerge at the A-exciton resonance (as for the case of WSe2 and WS2) or the B-exciton resonance (as for the case of MoSe2) depending on the sign of and the consequent ordering of the CBs. For hole-doped TMD monolayers, hexcitons should always emerge at the B-exciton, as investigated here for MoSe2 and as suggested by earlier spectroscopic data from both WSe2 and MoSe2 Wang:2017NL ; Liu:2021 . To the best of our knowledge, this has not yet been studied in hole-doped WS2 or MoS2. As a final point of discussion, we note that electron-doped MoS2 monolayers represent an interesting case: While early theory suggested that was small and negative (implying a CB ordering similar to MoSe2 Kormanyos:2015 ), more recent experimental work indicates an opposite CB ordering Robert:2020 ; Park:2022 , particularly when exciton effects are taken into account, making it more akin to that of WS2 and WSe2. In this case, a hexciton resonance can be expected to emerge in electron-doped MoS2 at the A-exciton, at an energy below that of the conventional charged excitons. Indeed, various recent studies have revealed additional optical resonances emerging in electron-doped MoS2 monolayers Klein:2021 ; Klein2:2021 ; Roch:2019 , although in some cases it was associated with exotic ferromagnetic order. Looking forward, we anticipate that composite 6-particle hexcitons (and, in high magnetic fields, 8-particle oxcitons) can provide a rich platform to study novel many-body effects and inter-valley correlations within a strongly interacting exciton-Fermi sea.
We thank X. Marie for helpful discussions, and we acknowledge support from the Los Alamos LDRD program and the US Department of Energy (DOE) “Science of 100 T” program. The National High Magnetic Field Lab is supported by National Science Foundation DMR-1644779, the State of Florida, and the US DOE. Work at the University of Rochester was supported by the DOE Basic Energy Sciences, Division of Materials Sciences and Engineering under Award No. DE-SC0014349.
References
- (1) G. Wang, A. Chernikov, M. M. Glazov, T. F. Heinz, X. Marie, T. Amand, and B. Urbaszek, Excitons in atomically thin transition metal dichalcogenides, Rev. Mod. Phys. 90, 021001 (2018).
- (2) T. Mueller and E. Malic, Exciton physics and device application of two-dimensional transition metal dichalcogenide semiconductors, npj 2D Materials 2, 29 (2018).
- (3) A. Splendiani, L. Sun, Y. Zhang, T. Li, J. Kim, C.-Y. Chim, G. Galli, and F. Wang, Emerging Photoluminescence in Monolayer MoS2, Nano Lett. 10, 1271 (2010).
- (4) K. F. Mak, C. Lee, J. Hone, J. Shan, and T. F. Heinz, Atomically Thin MoS2: A New Direct-Gap Semiconductor, Phys. Rev. Lett. 105, 136805 (2010).
- (5) Y. Li, A. Chernikov, X. Zhang, A. Rigosi, H. M. Hill, A. M. van der Zande, D. A. Chenet, E.-M. Shih, J. Hone, and T. F. Heinz, Measurement of the optical dielectric function of monolayer transition-metal dichalcogenides: MoS2, MoSe2, WS2, and WSe2, Phys. Rev. B 90, 205422 (2014).
- (6) J. S. Ross et al., Electrical control of neutral and charged excitons in a monolayer semiconductor, Nat. Commun. 4, 1474 (2013).
- (7) K. F. Mak, K. He, C. Lee, G. H. Lee, J. Hone, T. F. Heinz, and J. Shan, Tightly bound trions in monolayer MoS2, Nat. Mater. 12, 207-211 (2013).
- (8) Z. Wang, L. Zhao, K. F. Mak, and J. Shan, Probing the Spin-Polarized Electronic Band Structure in Monolayer Transition Metal Dichalcogenides by Optical Spectroscopy, Nano Lett. 17, 740-746 (2017).
- (9) K. Kheng, R. T. Cox, Merle Y. d’Aubigne, F. Bassani, K. Saminadayar, and S. Tatarenko, Observation of negatively charged excitons in semiconductor quantum wells, Phys. Rev. Lett. 71, 1752 (1993).
- (10) G. V. Astakhov, D. R. Yakovlev, V. P. Kochereshko, W. Ossau, W. Faschinger, J. Puls, F. Henneberger, S. A. Crooker, Q. McCulloch, D. Wolverson, N. A. Gippius, and A. Waag, Binding energy of charged excitons in ZnSe-based quantum wells, Phys. Rev. B 65, 165335 (2002).
- (11) I. Bar-Joseph, Trions in GaAs quantum wells, Semicond. Sci. Technol. 20, R29-R39 (2005).
- (12) F. X. Bronold, Absorption spectrum of a weakly n-doped semiconductor quantum well, Phys. Rev. B 61, 12620 (2000).
- (13) R. A. Suris, V. P. Kochereshko, G. V. Astakhov, D. R. Yakovlev, W. Ossau, J. Nürnberger, W. Faschinger, G. Landwehr, T. Wojtowicz, G. Karczewski, and J. Kossut, Excitons and trions modified by interactions with a two-dimensional electron gas, Phys. Stat. Sol. B 227, 343-352 (2001).
- (14) R. A. Suris, Correlation Between Trion and Hole in Fermi Distribution in Process of Trion Photo-excitation in Doped QWs. In Optical Properties of 2D Systems with Interacting Electrons; Ossau, W., Suris, R., Eds; NATO Science Series (Kluwer, Dordrecht, 2003) pp 111-124. Online at: https://arxiv.org/abs/1310.6120
- (15) Y.-C. Chang, S.-Y. Shiau, and M. Combescot, Crossover from trion-hole complex to exciton-polaron in n-doped two-dimensional semiconductor quantum wells, Phys. Rev. B 98, 235203 (2018).
- (16) F. Rana, O. Koksal, C. Manolatou, Many-body theory of the optical conductivity of excitons and trions in two-dimensional materials, Phys. Rev. B 102, 085304 (2020).
- (17) M. Sidler, P. Back, O. Cotlet, A. Srivastava, T. Fink, M. Kroner, E. Demler, and A. Imamoglu, Fermi polaron-polaritons in charge-tunable atomically thin semiconductors, Nat. Phys. 13, 255-261 (2017).
- (18) D. K. Efimkin and A. H. MacDonald, Many-body theory of trion absorption features in two-dimensional semiconductors, Phys. Rev. B 95, 035417 (2017).
- (19) Y.-W. Chang and D. R. Reichman, Many-body theory of optical absorption in doped two-dimensional semiconductors, Phys. Rev. B 99, 125421 (2019).
- (20) M. M. Glazov, Optical properties of charged excitons in two-dimensional semiconductors, J. Chem. Phys. 153, 034703 (2020).
- (21) F. Rana, O. Koksal, M. Jung, G. Shvets, A. N. Vamivakas, and C. Manolatou, Exciton-trion polaritons in doped two-dimensional semiconductors, Phys. Rev. Lett. 126, 127402 (2021).
- (22) D. K. Efimkin, E. K. Laird, J. Levinsen, M. M. Parish, and A. H. MacDonald, Electron-exciton interactions in the exciton-polaron problem, Phys. Rev. B 103, 075417 (2021).
- (23) C. Fey, P. Schmelcher, A. Imamoglu, and R. Schmidt, Theory of exciton-electron scattering in atomically thin semiconductors. Phys. Rev. B 101, 195417 (2020).
- (24) E. Liu, J. van Baren, Z. Lu, T. Taniguchi, K. Watanabe, D. Smirnov, Y.-C. Chang, and C. H. Lui, Exciton-polaron Rydberg states in monolayer MoSe2 and WSe2, Nat. Commun. 21, 6131 (2021).
- (25) J. Li, M. Goryca, J. Choi, X. Xu, and S. A. Crooker, Many-Body Exciton and Intervalley Correlations in Heavily Electron-Doped WSe2 Monolayers, Nano Lett. 22, 426 (2022).
- (26) D. Van Tuan, S.F. Shi, X. Xu, S.A. Crooker, and H. Dery, Six-Body and Eight-Body Exciton States in Monolayer WSe2, Phys. Rev. Lett. 129, 076801 (2022).
- (27) D. Van Tuan and H. Dery, Composite excitonic states in doped semiconductors, Phys Rev. B 106, L081301 (2022).
- (28) C. Robert, T. Amand, F. Cadiz, D. Lagarde, E. Courtade, M. Manca, T. Taniguchi, K. Watanabe, B. Urbaszek, and X. Marie, Fine structure and lifetime of dark excitons in transition metal dichalcogenide monolayers, Phys. Rev. B 96, 155423 (2017)
- (29) E. Malic, M. Selig, M. Feierabend, S. Brem, D. Christiansen, F. Wendler, A. Knorr, and G. Berghauser, Dark excitons in transition metal dichalcogenides, Phys. Rev. Mater. 2, 014002 (2018).
- (30) M. He, P. Rivera, D. V. Tuan, N. P. Wilson, M. Yang, T. Taniguchi, K. Watanabe, J. Yan, D. G. Mandrus, H. Yu , H. Dery, W. Yao, and X. Xu, Valley phonons and exciton complexes in a monolayer semiconductor, Nat. Commun. 11, 618 (2020).
- (31) M. Yang, L. Ren, C. Robert, D. Van Tuan, L. Lombez, B. Urbaszek, X. Marie, and H. Dery, Relaxation and darkening of excitonic complexes in electrostatically doped monolayer WSe2: Roles of exciton-electron and trion-electron interactions, Phys. Rev. B 105, 085302 (2022).
- (32) M. Barbone et al., Charge-tuneable biexciton complexes in monolayer WSe2, Nat. Commun. 9, 3721 (2018).
- (33) Z. Li et al., Revealing the biexciton and trion-exciton complexes in BN encapsulated WSe2, Nat. Commun. 9, 3719 (2018).
- (34) S.-Y. Chen, T. Goldstein, T. Taniguchi, K. Watanabe, and J. Yan, Coulomb bound four- and five-particle intervalley states in an atomically-thin semiconductor. Nat. Commun. 9, 3717 (2018).
- (35) Y. Song and H. Dery, Transport theory of monolayer transition-metal dichalcogenides through symmetry, Phys. Rev. Lett. 111, 026601 (2013).
- (36) A. Kormányos, G. Burkard, M. Gmitra, J. Fabian, V. Zólyomi, N. D. Drummond, and V. Fal’ko, k.p theory for two-dimensional transition metal dichalcogenide semiconductors, 2D Mater. 2, 022001 (2015).
- (37) A. V. Stier, N. P. Wilson, K. A. Velizhanin, J. Kono, X. Xu, and S. A. Crooker, Magnetooptics of exciton Rydberg states in a monolayer semiconductor, Phys. Rev. Lett. 120, 057405 (2018).
- (38) J. Li, M. Goryca, N. P. Wilson, A. V. Stier, X. Xu, and S. A. Crooker, Spontaneous Valley Polarization of Interacting Carriers in a Monolayer Semiconductor, Phys. Rev. Lett. 125, 147602 (2020).
- (39) E. Courtade et al., Charged excitons in monolayer WSe2: Experiment and theory, Phys. Rev. B 96, 085302 (2017)..
- (40) A. Hichri and S. Jaziri, Trion fine structure and anomalous Hall effect in monolayer transition metal dichalcogenides, Phys. Rev. B 102, 085407 (2020).
- (41) A. M. Jones, H. Yu, N. J. Ghimire, S. Wu, G. Aivazian, J. S. Ross, B. Zhao, J. Yan, D. G. Mandrus, D. Xiao, W. Yao, and X. Xu, Optical generation of excitonic valley coherence in monolayer WSe2, Nat. Nanotech. 8, 634-638 (2013).
- (42) Z. Wang, J. Shan, and K. F. Mak, Valley- and spin-polarized Landau levels in monolayer WSe2, Nat. Nanotech. 12, 144-149 (2017).
- (43) T. Wang et al., Observation of Quantized Exciton Energies in Monolayer WSe2 under a Strong Magnetic Field, Phys. Rev. X 10, 021024 (2020).
- (44) T. Smoleński, O. Cotlet, A. Popert, P. Back, Y. Shimazaki, P. Knüppel, N. Dietler, T. Taniguchi, K. Watanabe, M. Kroner, and A. Imamoglu, Interaction-Induced Shubnikov–de Haas Oscillations in Optical Conductivity of Monolayer MoSe2, Phys. Rev. Lett. 123, 097403 (2019).
- (45) P. Kapuscinski et al., Valley polarization of singlet and triplet trions in a WS2 monolayer in magnetic fields, Phys. Chem. Chem. Phys. 22, 19155-19161 (2020).
- (46) M. Zinkiewicz et al., Excitonic complexes in n-doped WS2 monolayer, Nano Letters 21, 2519 (2021).
- (47) J. Zipfel, K. Wagner, J. D. Ziegler, T. Taniguchi, K. Watanabe, M. A. Semina, and A. Chernikov, Light-matter coupling and non-equilibrium dynamics of exchange-split trions in monolayer WS2, J. Chem. Phys. 153, 034706 (2020).
- (48) C. Robert et al., Spin/valley pumping of resident electrons in WSe2 and WS2 monolayers, Nat. Commun. 12, 5455 (2021).
- (49) M. Goryca, J. Li, A. V. Stier, T. Taniguchi, K. Watanabe, E. Courtade, S. Shree, C. Robert, B. Urbaszek, X. Marie and S. A. Crooker, Revealing exciton masses and dielectric properties of monolayer semiconductors with high magnetic fields, Nat. Commun. 10, 4172 (2019).
- (50) G. Plechinger, P. Nagler, A. Arora, A. Granados del Aguila, M. V. Ballottin, T. Frank, P. Steinleitner, M. Gmitra, J. Fabian, P. C. M. Christianen, R. Bratschitsch, C. Schüller, and T. Korn, Excitonic Valley Effects in Monolayer WS2 under High Magnetic Fields, Nano Lett. 16, 7899 (2016).
- (51) B. Scharf, D. van Tuan, I. Zutic, and H. Dery, Dynamical screening in monolayer transition-metal dichalcogenides and its manifestations in the exciton spectrum, J. Phys.:Cond. Matt. 31, 203001 (2019).
- (52) C. Robert et al., Measurement of the spin-forbidden dark excitons in MoS2 and MoSe2 monolayers, Nat. Commun. 11, 4037 (2020).
- (53) S. Park, S. Arscott, T. Taniguchi, K. Watanabe, F. Sirotti, and F. Cadiz, Efficient valley polarization of charged excitons in Molybdenum disulfide monolayers by optical pumping, Commun. Phys. 5, 73 (2022).
- (54) J. Klein et al., Trions in MoS2 are quantum superpositions of intra- and intervalley spin states. Phys. Rev. B 105, L041302 (2022).
- (55) J. Klein, A. Hötger, M. Florian, A. Steinhoff, A. Delhomme, T. Taniguchi, K. Watanabe, F. Jahnke, A. W. Holleitner, M. Potemski, C. Faugeras, J. J. Finley, and A. V. Stier, Controlling exciton many-body states by the electric-field effect in monolayer MoS2, Phys. Rev. Research 3, L022009 (2021).
- (56) J. G. Roch, G. Froelicher, N. Leisgang, P. Makk, K. Watanabe, T. Taniguchi, and R. J. Warburton, Spin-polarized electrons in monolayer MoS2, Nat. Nanotech. 14, 432-436 (2019).